Chapter 3 The Origin of Earth and the Solar System
Karla Panchuk
A solar system consists of a collection of objects orbiting one or more central stars. All solar systems start out the same way. They begin in a cloud of gas and dust called a nebula. Nebulae are some of the most beautiful objects that have been photographed in space, with vibrant colors from the gases and dust they contain, and brilliant twinkling from the many stars that have formed within them (Figure 3.3.1). The gas consists largely of hydrogen and helium, and the dust consists of tiny mineral grains, ice crystals, and organic particles.
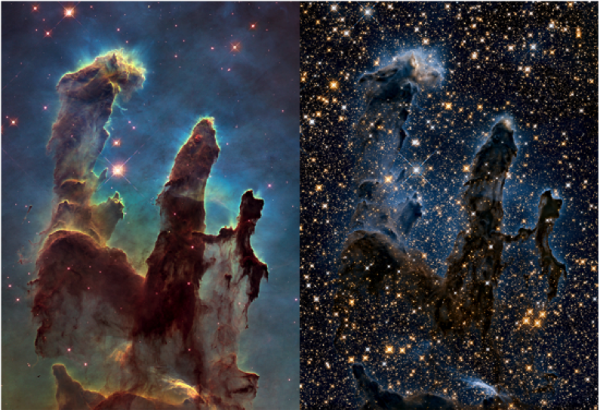
Step 1: Collapse a nebula
A solar system begins to form when a small patch within a nebula (small by the standards of the universe, that is) begins to collapse upon itself. Exactly how this starts isn’t clear, although it might be triggered by the violent behavior of nearby stars as they progress through their life cycles. Energy and matter released by these stars might compress the gas and dust in nearby neighborhoods within the nebula.
Once it is triggered, the collapse of gas and dust within that patch continues for two reasons. One of those reasons is that gravitational force pulls gas molecules and dust particles together. But early in the process, those particles are very small, so the gravitational force between them isn’t strong. So how do they come together? The answer is that dust first accumulates in loose clumps for the same reason dust bunnies form under your bed: static electricity. Given the role of dust bunnies in the early history of the solar system, one might speculate that an accumulation of dust bunnies poses a substantial risk to one’s home (Figure 3.3.2). In practice, however, this is rarely the case.
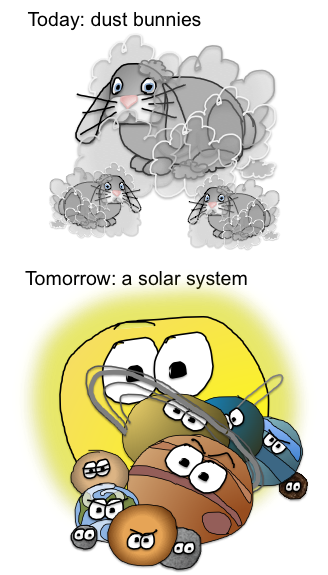
Step 2: Make a disk and put a star at its center
As the small patch within a nebula condenses, a star begins to form from material drawn into the center of the patch, and the remaining dust and gas settle into a disk that rotates around the star. The disk is where planets eventually form, so it’s called a protoplanetary disk. In Figure 3.3.3 the image in the upper left shows an artist’s impression of a protoplanetary disk, and the image in the upper right shows an actual protoplanetary disk surrounding the star HL Tauri. Notice the dark rings in the protoplanetary disk. These are gaps where planets are beginning to form. The rings are there because incipient planets are beginning to collect the dust and gas in their orbits. There is an analogy for this in our own solar system, because the dark rings are akin to the gaps in the rings of Saturn (Figure 3.3.3, lower left), where moons can be found (Figure 3.3.3, lower right).
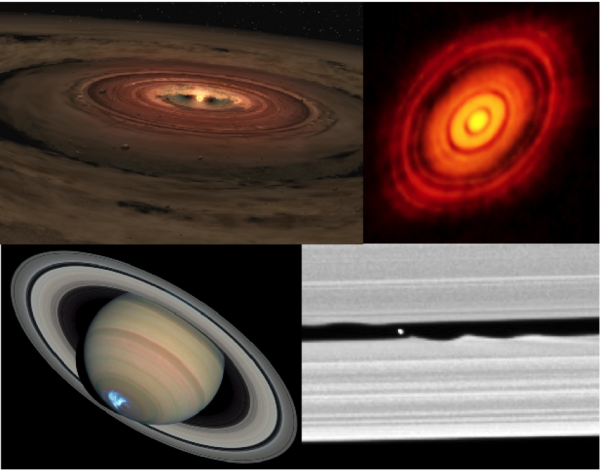
Step 3: Build some planets
In general, planets can be classified into three categories based on what they are made of (Figure 3.3.4). Terrestrial planets are those planets like Earth, Mercury, Venus, and Mars that have a core of metal surrounded by a mantle of rock. Jovian planets (also called gas giants) are those planets like Jupiter and Saturn that consist predominantly of hydrogen and helium. Ice giants are planets such as Uranus and Neptune that consist largely of water ice, methane (CH4) ice, and ammonia (NH3) ice, and have rocky cores. Often, the ice giant planets Uranus and Neptune are grouped with Jupiter and Saturn as gas giants; however, Uranus and Neptune are very different from Jupiter and Saturn.
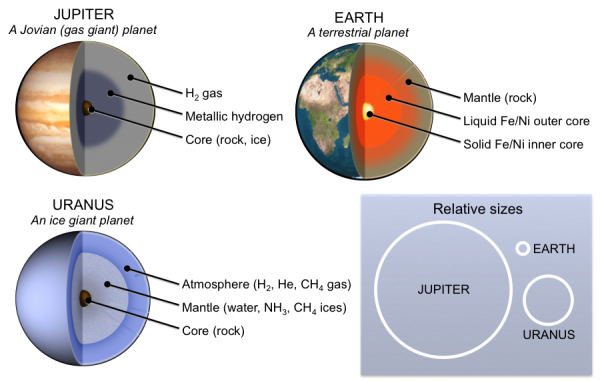
These three types of planets are not mixed together randomly within our solar system. Instead they occur in a systematic way, with terrestrial planets closest to the sun, followed by the Jovian planets and then the ice giants (Figure 3.3.5). Smaller solar system objects follow this arrangement as well. The asteroid belt contains bodies of rock and metal. Bodies ranging from metres to hundreds of metres in diameter are classified as asteroids, and smaller bodies are referred to as meteoroids. In contrast, the Kuiper belt (Kuiper rhymes with piper), and the Oort cloud (Oort rhymes with sort), which are at the outer edge of the solar system, contain bodies composed of large amounts of ice in addition to rocky fragments and dust. (We will talk more about smaller solar system objects in a moment.)
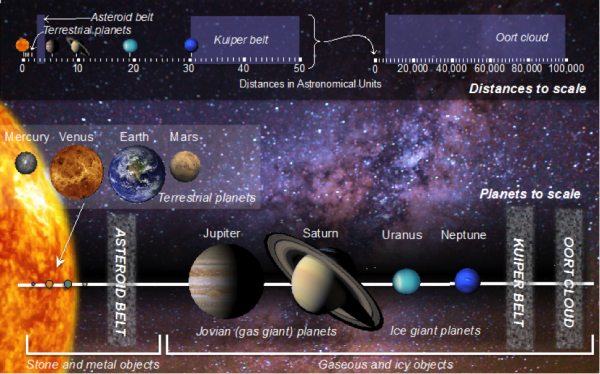
Part of the reason for this arrangement is the frost line (also referred to as the snow line). The frost line separated the inner part of the protoplanetary disk closer to the sun, where it was too hot to permit anything but silicate minerals and metal to crystalize, from the outer part of the disk farther from the Sun, where it was cool enough to allow ice to form. As a result, the objects that formed in the inner part of the protoplanetary disk consist largely of rock and metal, while the objects that formed in the outer part consist largely of gas and ice. The young sun blasted the solar system with raging solar winds (winds made up of energetic particles), which helped to drive lighter molecules toward the outer part of the protoplanetary disk.
The objects in our solar system formed by accretion. Early in this process, particles collected in fluffy clumps because of static electricity. As the clumps grew larger, gravity became more important and collected clumps into solid masses, and solid masses into larger and larger bodies. If you were one of these bodies in the early solar system, and participating in the accretion game with the goal of becoming a planet, you would have to follow some key rules:
- Keep your velocity just right. If you move too fast and collide with another body, you both smash up and have to start again. If you move slowly enough, gravity will keep you from bouncing off each other and you can grow larger.
- Your distance from the Sun will determine how big you can get. If you are closer, there is less material for you to collect than if you are farther away.
- To begin with, you can only collect mineral and rock particles. You have to grow above a certain mass before your gravity is strong enough to hang onto gas molecules, because gas molecules are very light.
- As your mass increases, your gravity becomes stronger and you can grab material from farther away. The bigger you are, the faster you grow.
You would also have to watch out for some dangers:
- In the early stages of the game, the protoplanetary disk is turbulent, and you and other objects can get thrown into different orbits or at each other. This might be a good thing, or it might not, depending on how the rules above apply to you.
- If the game progresses to the point where there is no more material within your reach and you are not yet a planet, then it’s game over.
- If you slow down too much (e.g., from bumping into other objects), you could spiral into the Sun (game over).
- If another planet gets big enough, it can:
- Rip you apart and then swing the pieces around so fast that for the rest of the game you collide too hard with other pieces to grow any bigger (game over)
- Fling you out of the solar system (game over)
- Grab you for itself (game over)
- Trap you in an orbit around it, turning you into a moon (game over, and incredibly humiliating)
The outcome of the game is evident in Figure 3.3.5. Today eight official winners are recognized, with Jupiter taking the grand prize, followed closely by Saturn. Both planets have trophy cases with more than 60 moons each, and each has a moon that is larger than Mercury. Prior to 2006, Pluto was also counted a winner, but in 2006 a controversial decision revoked Pluto’s planet status. The reason was a newly formalized definition of a planet, which stated that an object can only be considered a planet if it is massive enough to have swept its orbit clean of other bodies. Pluto is situated within the icy clutter of the Kuiper belt, so it does not fit this definition. Pluto’s supporters have argued that Pluto should have been grandfathered in, given that the definition came after Pluto was declared a planet, but to no avail. Pluto has not given up, and on July 13, 2015, it launched an emotional plea with the help of the NASA’s New Horizons probe. New Horizons sent back images of Pluto’s heart (Figure 3.3.6). On closer inspection, Pluto’s heart was discovered to be broken.
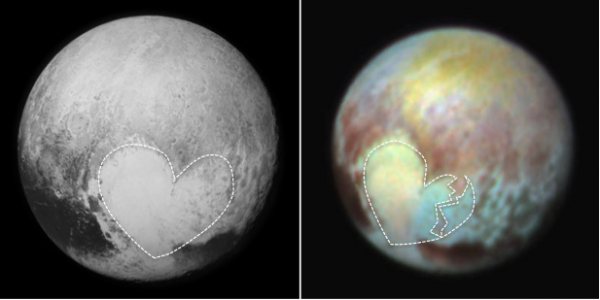
The rules and dangers of the planet-forming game help to explain many features of our solar system today.
- Proximity to the Sun explains why the terrestrial planets are so much smaller than the gas giant and ice giant planets.
- Mars is smaller than it should be, given the rule that distance from the Sun determines how much material a body can accumulate, and this can be explained by its proximity to Jupiter. Jupiter’s immense gravity interfered with Mars’ ability to accrete. Further evidence of Jupiter’s interference is the debris field that forms the asteroid belt. From time to time, Jupiter still flings objects from the asteroid belt out into other parts of the solar system, some of which have collided with Earth to catastrophic effect.
- The Kuiper belt is an icy version of the asteroid belt, consisting of fragments left over from the early solar system. The material in the Kuiper belt is scattered because of Neptune’s gravity. From time to time, Jupiter interferes here as well, flinging Kuiper belt objects toward the Sun and into orbit. As these objects approach the Sun, the Sun causes dust and gas to be blasted from their surface, forming tails. We know these objects as comets.
- Comets may also come from the Oort cloud where gravitational forces from outside of the solar system can hurl objects from the Oort cloud toward the Sun.
Exercise 3.1 How do we know what other planets are like inside?
The densities of planets give us important clues about the planets’ compositions. For example, in our solar system, Earth (a terrestrial planet) has a density of 5.51 grams per cubic centimetre (g/cm3), but Jupiter (a gas giant) has a density of 1.33 g/cm3. We can also use density to determine something about the interior structures of planets. In this exercise, you will determine how much of each terrestrial planet is made up of core, and translate that result to a diagram for easy comparison.
It is useful to approximate the structure of a terrestrial planet as having two parts: a metal core and a rocky mantle. If we know the density of the planet as a whole, and the densities of the materials making up the rocky mantle and the core, we can find out how much of the planet is core and how much is rocky. The density of the planet is the sum of the percent having the density of the core and the percent having the density of rock. This can be written as follows:
planet density = % core/100 x core density + (1− %core ÷ 100) × rock density
Rearranging the equation gives us:
% core = (planet density − rock density)/ (core density − rock density) × 100
Step 1.
Find the percent core for each of the terrestrial planets using the data in Tables 3.1 and 3.2. For our calculations, the planet density will be the uncompressed density of the planet. Uncompressed density is the density after removing the effects of gravity squeezing the planet together. (Notice that the density we mentioned for Earth is 5.51 g/cm3, but Earth’s uncompressed density is only 4.05 g/cm3.) The first one is done for you.
Description | Density (g/cm3) | Source | Why? |
---|---|---|---|
Core density | 8.00 | iron meteorites | Iron meteorites come from the cores of broken up asteroids and planets and approximate what the density of Earth’s core would be without gravitational squeezing. |
Rocky mantle density | 3.25 | HED* stony meteorites | HED (Howardites, Eucrites, and Diogenites) meteorites come from the rocky mantles of asteroids and planets that have separated into mantle and core, and then broken up. These approximate what the density of Earth’s mantle would be without gravitational squeezing. |
*HED stands for the names of three types of meteorites: howardites, eucrites, and diogenites.
Description | Earth | Mars | Venus | Mercury |
---|---|---|---|---|
Planet density (uncompressed)
in g/cm3 |
4.05 | 3.74 | 4.00 | 5.30 |
Percent core
((planet density − 3.25 g/cm3) ÷ 4.75 g/cm3 ) × 100 |
16.8% |
Step 2.
Once we have the percent of core, we can use it to find the volume of the core for each planet. The core volume is the percent of core times the volume of the planet. Use the planet volumes in Table 22.3 to calculate the core volume. Record your answers.
Description | Earth | Mars | Venus | Mercury |
---|---|---|---|---|
Planet volume* in km3 | 1.47 × 1012 | 1.72 × 1011 | 1.22 × 1012 | 6.23 × 1010 |
Core volume in in km3 (% core ÷ 100) × planet volume |
2.48 × 1011 |
*Unsqueezed values
Step 3. We can get the radius of the core from its volume by using the formula for the volume of a sphere (volume = 4 ÷ 3pr3, where r is the radius). This calculation is done for you in Table 22.4. From these values, express each radius as a percentage of the total radius. To do this, divide the core radius by the planet radius and multiply by 100. Using your results, fill in the diagrams at the bottom of Table 3.4 by drawing in the boundary between the core and mantle.
One of the terrestrial planets is thought to have been involved in collisions that resulted in the permanent loss of a substantial amount of its mantle. You might be able to guess which one it is from the uncompressed densities of the planets. It should also be clear from your diagrams. Which planet is it?
See Appendix 3 for Exercise 3.1 answers.
Media Attributions
- Physical Geology-2nd Edition, by Steven Earle is licensed under CC BY 4.0, chapter written by Karla Panchuk.
- Figure 3.3.1: “A View of the Pillars of Creation in Visible and in Near-Infrared Light” by NASA, ESA, and the Hubble Heritage Team (STScI/AURA). Public domain.
- Figure 3.3.2: © Karla Panchuk. CC BY. Inspiration from NASA/JPL.
- Figure 3.3.3 (upper left): “Artist’s impression of a disc forming into a solar system around a red dwarf” by NASA/JPL-Caltech/T. Pyle.
- Figure 3.3.3 (upper right): “HL Tauri” © ALMA (ESO/NAOJ/NRAO). CC BY.
- Figure 3.3.3 (lower left): “Saturn Aurora – January 26, 2004” by NASA, ESA, J. Clarke (Boston University), and Z. Levay (STScI). Public domain.
- Figure 3.3.3 (lower right): “Moon Daphnis S2005 S1” by NASA/JPL/Space Science Institute. Public domain.
- Figure 3.3.4: © Karla Panchuk. CC BY. After public domain images by FrancescoA and NASA (Image 1, Image 2).
- Figure 3.3.5: © Karla Panchuk. CC BY-SA. Includes the following images: Planet photographs by NASA and Milky Way photo by ForestWanderer
- Figure 3.3.6: © Karla Panchuk. CC BY. Based on NASA/APL/SwRI.
a star and the planets surrounding it
a cloud of interstellar dust and gases
a rotating cloud of gas and dust surrounding a young star
a planet with a rocky mantle and crust and metallic core (e.g., Earth)
a gas giant
a large planet composed mostly of hydrogen and helium (e.g. Jupiter)
a planet that is comprised mainly of gases heavier than hydrogen and helium, including oxygen, carbon, nitrogen, and sulfur (e.g., Uranus and Neptune)
the region between the orbits of Mars and Jupiter that is populated with many asteroids
a rocky body orbiting the Sun
a fragment of either stony or metallic debris in space
a region of the Solar System beyond the orbit of Neptune that is populated by small objects and dwarf planets (including Pluto)
a spherical cloud of icy objects extending from between about 5,000 and 500,000 astronomical units (Sun-Earth distances) from the Sun (thought to be the source area of comets)
in the context of planetary systems the boundary beyond which volatile components (e.g., water, carbon dioxide, methane, ammonia etc.) are frozen
in astronomy the radius around a star at which represents the boundary between gases (or liquids) and solids
a stream of ionized (charged) particles away from the Sun
the process by which solid celestial bodies are added to existing bodies during collisions
the density of planetary material that it would have it was not compressed by the planets gravitational force